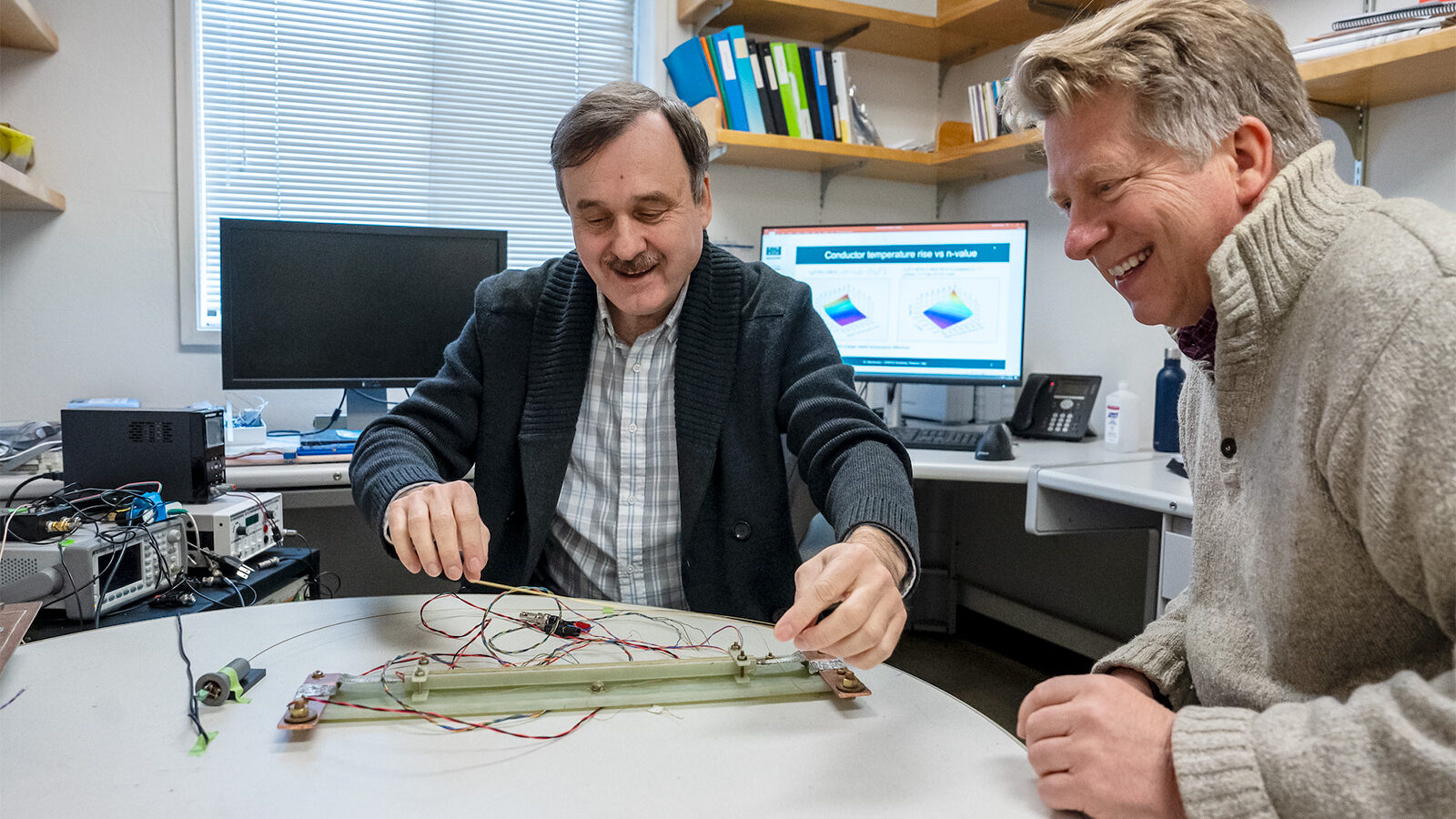
The particle accelerators that enable high-energy physics and serve many fields of science, such as materials, medical and fusion research, are driven by superconducting magnets that are, to put it simply, quite finicky.
Superconductors are a special class of materials which, when cooled below a certain temperature, carry large electrical currents without resistance. If you arrange the material in coils, the current passing through will produce strong magnetic fields, effectively storing the potential energy of the moving electrons in that form. But if they get too hot—only a few degrees above negative 452 Fahrenheit (4.2 Kelvin), or the temperature of liquid helium—they can suddenly regain their electrical resistance and dissipate the energy of the magnetic field in a rapid burst of heat.
A newer type of superconductor, known as a high-temperature superconductor, is poised to usher in another revolution for science and technology. High-temperature superconductors, or HTS, have the potential to produce even higher magnetic fields while operating at easier to maintain temperatures than traditional superconducting magnets.
In the new HTS materials, these unwanted heating events, known as “quenches,” are particularly costly, as they can destroy the magnet, damage nearby components and deplete significant volumes of the precious liquid coolants used to chill the magnet. Due to their powerful properties, these magnets are a hot topic of research and development, but protecting them from destructive events is a major hurdle to their broad application. The best solution would be to design HTS magnets that do not quench in the first place.
That’s what researchers at Lawrence Berkeley National Laboratory are working on.
Maxim Marchevsky and Soren Prestemon of Berkeley Lab’s Accelerator Technology & Applied Physics Division have developed a strategy to identify conditions under which HTS magnets can safely operate without the risk of a sudden heat build-up causing the magnet to fail.
“This is somewhat akin to designing a plane to enable safe landing in the case of engine failure, as opposed to designing the plane to survive a crash,” says Prestemon, who is Deputy Director of Technology for the ATAP Division.
Their work was published recently in Superconductor Science and Technology.
Because HTS magnets can tolerate higher density of the electric current and a wider range of temperatures while still acting as a superconductor, they are less prone to quenching than their low-temperature counterparts. However, detecting an oncoming quench is harder in HTS magnets because the superconducting properties switch off in very small pockets of the material. This means that the enormous magnetic energy of the coil is converted to heat across a small area, causing the temperature to rapidly rise to extremes in that location.
Such a loss in superconductivity is typically caused by the current surpassing the capacity of the superconductor, for example, due to imperfections in the material’s structure, or by increased heat caused by either a cooling system malfunction or an impact to the magnet by errant fast-moving particles from the accelerator or fusion reactor. Either way, the resulting quench is harder to monitor and may reach the point of no return faster than existing mitigation systems like coolant sprays can be activated.
Fortunately, several decades of HTS research and development has revealed that these materials can tolerate minor build-up of heat but stay in superconductor mode. Using this knowledge, Marchevsky and Prestemon realized they could calculate a window of operational parameters in which the HTS conductor will work without ever spiraling out of control into a quench.
“Because of that, we can actually address the problem differently. We can look for a sign of heat somewhere in the magnet, and if we detect it early enough, we can safely run down the current without actually quenching the magnet,” says Marchevsky, a staff physicist in ATAP.
The scientists’ theoretical work was validated with experiments using tape-shaped samples of Bi-2223 HTS material (a compound of bismuth, strontium, calcium, copper and oxygen) that were supplied with high current in an environment where minute temperature fluctuations could be detected and compared to the numerical predictions. The next step will be to test their approach on actual coils wound with HTS conductor material to replicate the form they would take inside particle accelerators and devices like MRI machines.
To successfully detect the pre-quench state in these coils, the scientists plan to use highly sensitive temperature monitoring systems developed by themselves and their colleagues in ATAP, a group with deep expertise in fundamental and applied accelerator magnet science. “There will be some challenges because we need to have distributed measurement of temperature, but that’s something we’ve been working on quite a lot in the past several years,” Marchevsky says.
He notes that traditional quench-detecting systems for low-temperature magnets monitor resistance across the magnet, which doesn’t work well for HTS magnets. “Various new techniques are being investigated and embedded in our real prototype magnets.”
Their techniques include ultrasonic-based, radiofrequency-based, and fiber optic-based sensor systems. The latter approach is the primary candidate for use in experimental plasma fusion energy reactors, which are one of the first real-world applications of HTS magnets on the horizon. Plasma fusion reactors need powerful magnets to confine mixtures of super-heated charged particles in a small space, and HTS magnets look promising to enable a breakthrough in this field.
Marchevsky and Prestemon hope that distributed temperature systems monitoring the entire magnet will be able to alert operators if any region is approaching the upper end of the safe temperature window. Then, the current delivered to the magnet can be reduced and quenching avoided.
If successful, the approach could enable widespread adoption of HTS magnets, ultimately leading to much higher magnetic fields and magnet systems that are cheaper to maintain than their low-temperature counterparts. These savings would help lower the costs of all accelerator-driven research and aid the moonshot goal of fusion energy.
“The fundamental science and precision diagnostics combined in this work exemplify the unparalleled set of ‘mesoscale to magnet’ capabilities the Lab brings to the drive for high temperature superconductors as transformative technologies across accelerators, fusion and applications,” says Cameron Geddes, ATAP Division Director.
Editor’s note: A version of this article was originally published by Lawrence Berkeley National Laboratory.